Another wobble method delivered the first new extrasolar planets. It relies on the influence of the Doppler effect on the spectrum of light coming from a star. The Doppler effect, as described in 1842 by Austrian physicist Christian Doppler, is something you are sure to have experienced. It happens anytime an object is moving and emitting waves at the same time. Sounds are waves in the air that we hear, so the sound of a passing car (or an ambulance siren) changes its pitch
because of the Doppler effect: you hear a slightly higher sound as the ambulance approaches, and a slightly lower one after it passes by. Moreover, it is the relative motion that matters. So if the ambulance is stopped with its siren on and you drive by it, you'll experience exactly the same Doppler effect. The Doppler effect can be used in a practical way to measure the speed of objects, as, for example, when police radar catches you speeding on the highway, or when meteorologists measure shifting wind speeds in an attempt to detect tornadoes.
Light also consists of wavesâelectromagnetic wavesâthat are shorter than sound waves. So, light is subject to the same Doppler effect as sound, and we can measure the relative speeds of stars, galaxies, and more. This is of huge importance to astronomy, where we have no way to approach these distant objects and measure their motions.
To use the Doppler effect, scientists employ a telescope, a prism, and a camera to record the light spectrum of a star. Then they look for a marker in the spectrum. The marker will be shifted to longer wavelengths (toward the red light) if the star in question is moving away from youâthe faster the star is moving, the more the marker is shifted. If the star is approaching you, the reverse is true. The majority of the visible light coming from a star is just like what we get from the hot filament of an incandescent lightbulb, and when dispersed into a spectrum it looks like a rainbow. But when a scientist looks at it with a prism, for example, she sees lots of markers. In the spectra of most stars these markers are narrow
dark lines which indicate that light at specific wavelengths is missing. These linesâspectrum absorption linesâare caused by atoms and ions near the star's surface. When light passes through a gas made of atoms, for example, some of it gets absorbed by the atoms; we see this every day with clouds. However, our eyes are not equipped to see that the light is also absorbed in numerous specific wavelengths, or colors, of that light. These absorption lines and their corresponding wavelengths are due to the electrons that orbit atoms (and ions and molecules). Electrons have numerous, strictly defined states of energyâdifferent orbits, if you willâand that is where light gets lost. Because these energy states are strictly defined, so are the absorption lines and their wavelengths. Therefore each atom of every known elementâfrom hydrogen to the heaviest known metalsâhas an unmistakable fingerprint consisting of thousands of absorption lines all over the spectrum.
These spectrum absorption lines are the markers we use to measure the Doppler effect in stars. In doing so, we can discern very small changes in the speeds of stars, including the wobble that an orbiting planet would cause. What is more, the Doppler effect measured this way will allow us to measure the actual speed of the star on
its own
orbit around the center of mass. That orbital speed, together with the orbital periodâwhich is the same for star and planet as they revolve around their center of massâwill allow us to measure their masses. We know how to estimate the mass of a star by measuring its spectrum; these absorption lines carry
a lot of information. Therefore, we can determine the mass of the planet. There is one small hitch: we can't measure the angle at which the orbit is inclined toward us, as the Doppler effect gives us relative motion only. Consequently some uncertainty about the mass of the planet remains. To remove that uncertainty, we need to turn to other methods of planet discovery and studyâfor example, the transit method (more on that in the next chapter).
The third practical way to detect the wobble is by timing. If there is a strictly periodic signal we can measure and time with a precise clock, the wobble due to a planet will appear as a cyclic variation in the period of that signal. What could such a periodic signal be? Well, it could come from a pulsarâa neutron star spinning very quickly and emitting radio pulsesâor from two stars orbiting very close to each other and eclipsing periodically (every few hours or days). That is how in 1992 the planets orbiting the pulsar PSR 1257+12 were discovered by the radio astronomers A. Wolszczan and D. Frail.
6
This was a remarkable discovery, but it did not gather the attention lavished on the 1995 discovery of 51 Peg b for two reasons. First, the pulsar planets were exoticâboth as planets and as a planetary system.
7
Second, it turns out that pulsar planets are extremely rare. With only a couple of systems known, there is not much to study and little to help us understand their origin.
The timing technique can be used also when one planet is pushed and pulled around by a second planet. For example, if we have a system with a hot Jupiter and keep observing the
hot Jupiter as it regularly orbits its star (e.g., by marking events like eclipses or transits; see the next chapter), we can catch variations in its regular orbit caused by an unseen second planet.
8
In some cases, the second planet is easier to catch by such timing variations, than by seeing the corresponding wobble of the star. This technique has become one of the big early science successes of the Kepler planet search mission. For example, five transiting planets in the Kepler-11 system could be confirmed and their masses derived by using transit timing variations alone.
9
There is another method of planet discovery that also exploits the favorable star-to-planet mass ratioâgravitational lensing, an effect predicted by, and famous for its help in confirming, Einstein's general theory of relativity. The pioneering theoretical work of the late Princeton astrophysicist Bohdan Paczynski showed the practical uses of Einstein's prediction and led to the creation of several international projects to monitor stars for gravitational lensing.
10
For this, a source of light is needed, usually another star, behind the star being investigated. As we look at it, the light from the background star will be bent by the gravity of the intervening star. If the intervening star has an attendant planet, this will alter the lensing effect in a noticeable way.
11
In 2005 J. P. Beaulieu and his team discovered a planet with about five to six times the mass of Earth, which we call a super-Earth.
12
The planet bears the impossibly complex name OGLE-2005-BLG-390Lb, and it orbits a small star at a distance at least two to three times greater than the distance at which our planet orbits the Sun.
13
That is probably as much as we will ever learn about planet OGLE-2005-BLG-390Lb, because the gravitational lensing method allows just a single glimpse, a sort of a snapshot. By its nature the observation cannot be repeated.
14
However, the value of the gravitational lensing method to extrasolar planets is in the statistics. In essence, the method is a general scanning approach that allows the discovery of super-Jupiters and super-Earths on an equal footing. So, even though very few planets have been discovered with this method so far, it was possible to notice a statistical trend that smaller planets are at least as common as giant planets, and probably are even more numerous.
15
The first decade of extrasolar planets saw the maturation of several methods of discovery. Some of them complement each other and also help us study the planets we discover. This is crucial in our quest to find out if the Solar System, planet Earth, andâultimatelyâEarth life, are unique, rare, or common in the Universe. Once we have achieved that, we will have completed the Copernican revolution. The last of these, which exploits the size ratio of planets and stars, and is known as the transiting method, seems to be the easiest. After all, the inequality between star and planet is the least daunting, with a factor 10 to 100 difference in size. And indeed, in our quest, transiting plays a special role. But, as often happens, there is a catch.
CHAPTER FOUR
CHASING TRANSITS
I
t is about 5:30 in the morning and I am racing down an empty avenue by the Charles River in Cambridge, Massachusetts. Crossing the bridge into Harvard Square, I keep an anxious eye on the eastern horizon, where the rising sun is competing with clouds for a piece of the sky. My destinationâthe Harvard University Science Center, just north of Harvard Yardâis a bizarre scene. By the entrance, the Harvard marching band plays an obscure piece over and over again, as hundreds of people try to get to the roof. Everyone is here to see a sight not seen by humankind for 122 years. It is June 8, 2004, and a transit of Venus is under way.
1
The transitâduring which the little black disk of Venus passes in front of the big glowing disk of the Sunâis one of those spectacles in the sky that lets you “see” the inner Solar System as if it were a mobile. Transits of Venus are spectacular
but rare: only every
other
generation can witness them. This generation is lucky: the transits of Venus now happen in pairs and the next one will occur on June 6, 2012.
2
Mark your calendar!
Today the transit of Venus is of little value to the research astronomer, but two transit cycles ago, back in the year 1769, the scientific rewards of witnessing such a transit were great, and international efforts to observe it were remarkable. According to William Sheehan and John Westfall, these efforts represented an eighteenth-century equivalent of the twentieth-century race to the Moon.
3
At stake was a unique opportunity to use the passage of Venus in front of the Sun to measure precisely the distance between Earth and the Sun, and thus distances across the Solar System. This was important for more than pure science, as the British Transit Committee duly noted in a memo to King George III in 1767; it was also crucial for navigation. That was enough to convince the king to support a mission. On May 25, 1768, Captain James Cook was appointed to do the job, and his famous trip to the South Pacific ensued.
A transit is in essence an eclipse of the Sun by either Mercury or Venus, although the event is not nearly as dramatic as when the Moon eclipses the Sun. Planetary transits do little to diminish the amount of sunlight we seeâjust a small fraction of a percent, too tiny for us to noticeâbut they do form a black dot against the Sun as they pass between us and the star. Historically, the term “transit” was reserved for Mercury and Venus, but these days it finds a much wider application in the hunt for planets around other stars. As with
Mercury or Venus, the planet will obscure only 1 percent or less of its surface (see
Figure 4.1
).
Â
FIGURE 4.1
.
schematic illustration of a transiting planetary system. The transiting planet is shown in three different positions on its orbit. When in transit, the dark night side of the planet is facing us, but we can glimpse its atmosphere in the stellar light that passes through it. When the planet is on the side (left side), it shows phases to us, just as our Moon does. Half an orbit after a transit, the planet will pass behind its starâknown as occultation or eclipse.
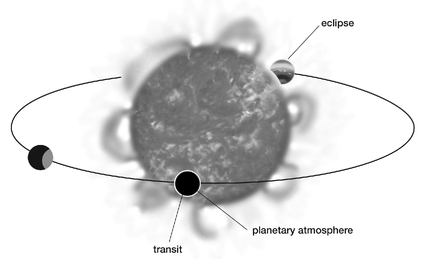
As it passes across the disk of its parent star, a planet will dim the star's light by a fraction equal to its projected area compared to the star's shining projected area. The area of a circle depends on its radius,
r,
squared. Therefore the star's light will dim by a fraction (
r
p
/
r
s
)
2
, where
r
p
and
r
s
are the respective radii of the planet and star. For a planet the size of Jupiter and a star the size of the Sun, this dimming effect would be roughly 1 percent, which is easily detectable even with amateur equipment.
4
Earth, however, some 109 times smaller than the Sun, will dim just 1/(109)
2
= 0.008 percent of the Sun's light when transiting. That is challenging to see, but not impossible.
The transiting method for planet discovery works because the dimming due to a planet transit can be measured by using the brightness of the star, known as photometry (measuring photons). Photometry is different from spectroscopy, the measurement of the color of light; for one thing, you need a camera to do photometry, and most often that camera is attached to a smaller telescope. The main difficulty is that a transit requires the planet's orbit to be almost exactly edge-on as we look at the star, which is very unlikely, as planetary systems in our Galaxy are inclined randomly in all possible directions (
Figure 4.2
).
5
Therefore, from our own vantage point, the probability that we'll see a transiting planet among the ones that are out there will be equal to the ratio of the stellar radius to the size of the planet's orbit.
6
This is generally less than 1 percent.